Vol 6 No 1 2021 – 9
INVESTIGATION / RESEARCH
Chemical Pretreatments on Residual Cocoa Pod Shell Biomass for Bioethanol Production
1Jose F. Alvarez-Barreto*, 1 Fernando Larrea, 1 Maria C. Pinos, 1 Jose Benalcázar, 1 Daniela Oña, 1Carolina Andino, 1Daniela A. Viteri, 2Marco Leon, 1Daniela Almeida-Streitwieser.
Available from: http://dx.doi.org/10.21931/RB/2020.06.01.9
ABSTRACT
Cocoa pod shell is an essential agricultural residue in Ecuador, and this study addressed its potential valorization for bioethanol production. For this, three types of pretreatments, acid, alkaline, and autohydrolysis, were applied to pod shells from two different cocoa types, national and CCN-51. to remove the lignin. Untreated and treated biomasses were characterized by composition, thermal stability, Fourier transformed infrared spectroscopy (FITR), and scanning electron microscopy (SEM). The treated biomass was then enzymatically hydrolyzed with cellulase. Reducing sugars were quantified after pretreatments and enzymatic hydrolysis, and the pretreatment liquors and the enzymatic hydrolysates were subjected to alcoholic fermentation with Saccharomyces cerevisiae.
There were substantial differences in composition between both biomasses, particularly in lignin content, with national cocoa having the lowest values. All pretreatment conditions had significant effects on biomass composition, structure, and thermal properties. After alkaline pretreatment, the biomass presented the highest cellulose and lowest lignin contents, resulting in the highest reducing sugar concentration in the pretreatment liquor. The highest lignin content was found after the acid pretreatment, which resulted in low, reducing sugar concentrations. Autohydrolysis produced similar results as the acid pretreatment; however, it resulted in the highest sugar concentration after enzymatic hydrolysis, while the acid-treated sample had negligible levels. After fermentation, there were no differences in productivity among the pretreatment liquors, but autohydrolysis had the largest ethanol yield. In the hydrolysates, it was also autohydrolysis that resulted in higher productivity and yield. Thus, there is an indication of the formation of inhibitors, both enzymatic activity and ethanol production, in the acid and alkaline pretreatments, and this should be tackled in future research. Nonetheless, given the crucial changes observed in biomass, we believe that cocoa pod shell pretreatment has potential for the generation of reducing sugars that could be further used in different bioprocesses, nor only bioethanol production.
Keywords: lignocellulosic biomass, bioethanol, cocoa, pretreatment, bioprocesses
INTRODUCTION
Currently, 83% of the world’s energy demand is satisfied by burning fossil fuels; The Intergovernmental Panel on Climate Change (IPCC) found that fossil fuels are the main generators of greenhouse gases, which accelerate global warming 1. Thus, there is urgency in developing alternative fuels, such as biodiesel from food waste 2; and second-generation bioethanol from residual lignocellulosic biomass 3.
The primary sources of lignocellulosic biomass are agricultural residues, wood, and energy crops. This biomass is mainly composed of cellulose (35-50%), hemicellulose (20-35%), lignin (10-25%), and, to a lesser extent, there are proteins, pectin, oils, and ashes 4. However, lignocellulosic biomass has a complex structure because cellulose is wrapped in a hemicellulose matrix, which, in turn, is surrounded by lignin walls. The latter is a rigid mesh because of the aromatic subunits in its macromolecular structure 5.
Studies have shown that implementing a biomass pretreatment is crucial to break down the lignin structure, eliminate hemicellulose, reduce the crystallinity of cellulose, and increase the available surface area for enzymes and microorganisms to act 6. Pretreatments are classified as physical, chemical, and biological, but they can also be combined to increase bioprocess performance and productivity of the desired product7.
A commonly used biological pretreatment of lignocellulosic biomass is enzymatic hydrolysis since it releases sugars that will later be transformed into ethanol thanks to fermentation8. However, low yields are observed due to the complex structure of lignocellulosic biomass. For this reason, before enzymatic hydrolysis, acidic and alkaline pretreatments have been used to remove lignin and hemicellulose and decrease the cellulose crystallinity 4.
Some biomasses have already been pretreated using these technologies and assessed for bioethanol production. Among the most studied sources are sugarcane bagasse, fruit waste, and water-insoluble fractions of sugar straw. In these studies, industrial yeast Saccharomyces cerevisiae for alcoholic fermentation has been used 9. Other less conventional sources, such as sweet sorghum, have also been explored, with promising results 10.
Cocoa pod husk represents a lignocellulosic residue poorly explored as raw material for bioethanol production. Ecuador is one of the largest cocoa producers in the world. In 2015, 236,677 thousand metric tons of cocoa beans were exported, where 47% corresponds to fine aroma cocoa, and the rest comes from a less flavorful but more productive clone called CCN-51 (Colección Castro Naranjal 51) 11. Moreover, according to the Bioenergetic Atlas of Ecuador, this translated into more than 2 million tons of waste, mostly derived from the pod shell12. Usually, these residues are used as organic compost; however, no study demonstrates their fertilizing capacity. Animal feed has also been explored, but the pod has theobromine, a hepatotoxic alkaloid, and can be harmful to animal health 13. For this reason, the cocoa pod shell, being a very abundant agricultural residue, can be used as a source of great importance for the production of bioethanol.
Therefore, the present work aims to evaluate the effects of different chemical pretreatments followed by enzymatic hydrolysis on biomass composition and properties and the generation of reducing sugars, both in the pretreatment liquors and enzymatic hydrolysates, and their potential use in alcoholic fermentation.
MATERIALS AND EXPERIMENTAL METHODS
Pod shells from two cocoa types were subjected to three different pretreatments: alkaline, acid, and autohydrolysis, and the liquors were stored. The resulting solids were then subjected to enzymatic hydrolysis, using cellulose. After that, alcoholic fermentations were carried out from the pretreatment liquors and hydrolysates. In Figure 1, the block diagram for obtaining ethanol from a cocoa pod shell is presented.
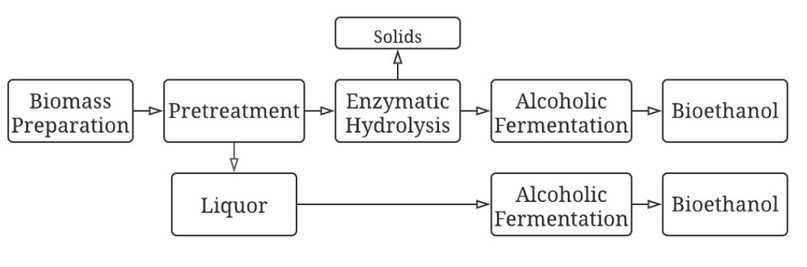
Figure 1. Block diagram for cocoa pod shell valorization to produce bioethanol.
Biomass preparation
Pod shells from the delicate aroma, national cocoa, and CCN-51 clone (Coleccion Castro Naranjal 51) were collected, thoroughly washed, cut into 1x1cm pieces, and dried at 60 °C until constant weight. Then, the dry sample was ground and sieved to a particle size range of 150 and 600μm.
Bioethanol production
Biomass Pretreatment
For each pretreatment, 1g of dry sample was pretreated with 15mL of solution for 4 hours at 100 ° C, under reflux, according to our laboratory’s preliminary results (Data not shown). The pretreated biomass was thoroughly rinsed in distilled water until neutral pH and dried at 40ºC, until constant weight. The pretreatment liquor was saved for fermentation and reducing sugar quantification. Pretreatments consisted of 2% w/v NaOH for the alkaline conditions, 2% v/v sulfuric acid for the acid attack, and distilled water for autohydrolysis 14.
Enzymatic hydrolysis
For the enzymatic hydrolysis, 7g of pretreated biomass were suspended in 50mL of sterile 50mM sodium citrate buffer solution, at pH 4.8, in an amber bottle. Cellulase (Rohament CL) was added at a concentration of 177 (ECU / mL). The bottle was then purged with N2 and capped. Hydrolysis was carried out at 55° C and 50rpm for 72 hours 15. The hydrolysate was separated through filtration for further fermentation, and the biomass was rinsed and dried as previously described for characterization.
Fermentation
In a 50mL conical tube, previously autoclaved, 30mL of the pretreatment liquor or hydrolysate was placed. Then, S. cerevisiae (RC212 yeast) was added at 8g/L and activated at 30 ° C for 20 minutes. Fermentation was carried out in a microbiological incubator at 30°C for 72 hours16. Finally, samples were taken from each of the fermenters after 24, 48, and 72 hours for sugar quantification.
Biomass Characterization
Composition
The untreated and pretreated biomass, moisture, fat, protein, and ash contents were determined according to standardized methods 17–20.
To determine cellulose content, the method reported by Dominguez et al. (2012) was followed. Briefly, 1g of dry sample was treated with 15mL of 80% acetic acid, along with 1.5mL of 68% nitric acid, for 20 min, under reflux. This was followed by filtration with hot water and small amounts of ethanol. As a next step, the sample was placed in crucibles and dried in the oven at 105°C for 24 hours. The sample was allowed to cool, weighed, and placed in the muffle for 5 hours at 540 ° C 21. Finally, the cellulose content was determined through equation (6).

Where, Wf, Wc, and Wi are the dry, calcined, and initial sample weight, respectively.
To determine lignin composition, 1 g of dry sample was suspended in 15mL of 72% sulfuric acid and stirred for 2 hours. The mixture was then mixed with 125mL of distilled water and boiled, in a reflux system, for 4 hours. Finally, the remaining solids were separated through filtration, washed with hot water and dried at 105 ° C for 3 hours 17. Lignin content was estimated through equation (7).

Where Wi and Wf are the initial sample weight and the weight after drying, respectively.
Physical-chemical characterization
To assess other significant compositional changes in the biomass due to pretreatment, Fourier transformed infrared spectroscopy (FTIR) was used. Spectra between 500-4000 cm-1 were obtained in a Cary 630 FTIR Spectrometer from Agilent Technologies. Meanwhile, changes in biomass morphology were assessed through Scanning Electron Microscopy (SEM). Untreated and pretreated biomasses were analyzed in a JEOL JSM-IT300 Scanning Electron Microscope at 50Pa and 20kV, using the MP-96040EXCS External Control Software for image processing.
The Thermal characterization was made to observe any changes produced in the biomass’s thermal stability after each pretreatment, as this is an indication of biomass composition and interaction among its components. Thermogravimetric analysis (TGA) was carried out in a PerkinElmer Simultaneous Thermal Analyzer STA 8000, where 5 mg of sample were heated from 25ºC to 600 ° C, with a heating ramp of 10 ° C / min. Nitrogen was used as inert gas with a flow of 20 ml/min.
Reducing Sugars and Ethanol Quantification
For reducing sugar quantification, the 3,5-dinitro-salicylic-acid (DNS) method was used 22, using a Hach Colorimeter DR / 870. A calibration curve was generated with solutions of known glucose concentrations.
To measure the ethanol obtained after each fermentation, the sample was initially centrifuged and filtered, followed by liquid-liquid extraction with dichloromethane, at a 2: 1 ratio. The organic phase was removed, and propanol was added, as an internal standard, at a 25:2 ratio. Finally, ethanol was quantified using gas chromatography in a GCMS-QP2010. Using a calibration curve, ethanol concentration was determined by relating the peak areas corresponding to ethanol and the internal standard. On the other hand, productivity was determined as grams of EtOH per kilograms of untreated biomass. While the yield was defined as the moles of EtOH divided by the moles of glucose present in the fermentation.
Statistical analyses
All analyzes and experiments were carried out in triplicates (n = 3). Results are reported as the (average ± standard deviation). Statistically significant effects were determined through an Analysis of Variance (ANOVA), and Tukey’s pairwise comparison was used to determine differences, with a 95% confidence level (p <0.05).
RESULTS AND DISCUSSION
Characterization of cocoa pod shells
Knowing the initial biomass composition is essential to know its variability regarding the cocoa type and assess the extent of changes caused by pretreatments. The proximal characterization of the cocoa pod shell for the two studied varieties is shown in Table 1.
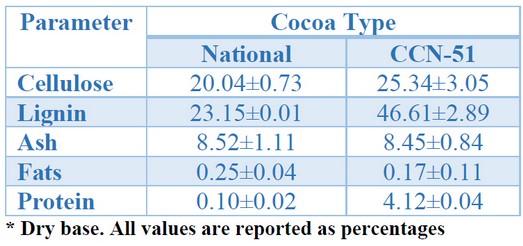
Table 1. Characterization of the pod shell from different cocoa types
Significant differences can be observed between national cocoa and CCN-51, perhaps being lignin content the most important. One of the primary purposes of biomass pretreatment is eliminating or altering lignin structure, and these differences can have significant repercussions on pretreatment conditions. In general, cocoa biomass composition is variable, as recognized in the literature. Campos-Vega reported the composition ranges of the cocoa pod’s shell, where protein content is between 2.1–9.1%, ash is between 5.8–13.0%, and the lignin content is 14.7–38.8% 23. This variability is related to the variety and irrigation, fertilizers used, transport, storage, soil, other factors 24, and crop’s climatic and environmental conditions 25.
Characterization of pretreated biomass
Pretreatment yields, defined as the ratio between the treated and untreated biomass, are essential for industrial applications. It would give a clear indication of the amount of residual pod shell needed to produce a desired amount of bioethanol. The values of pretreatment yield for each of the biomasses are presented in Figure 2.
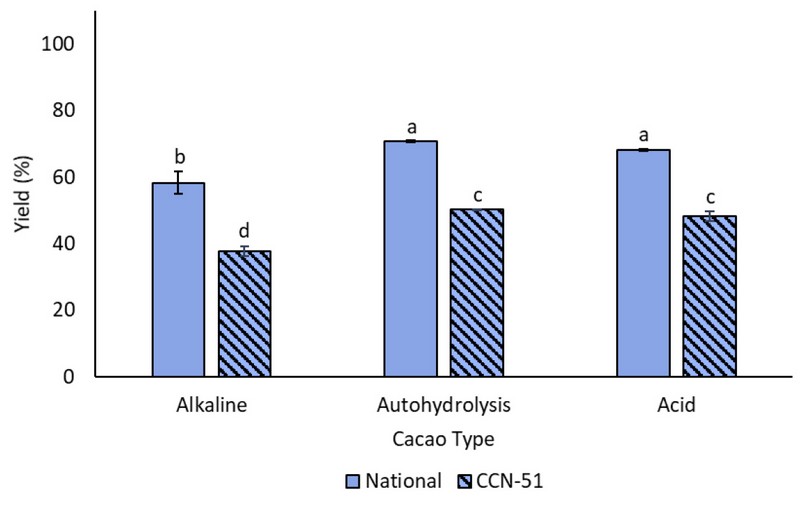
Figure 2. The yield of the pod shell, from different cocoa types, after each pretreatment. Pretreatments with the same letters do not present statistically significant differences
In general, it was found that, for all pretreatments, the yield was lower for CCN-51. In both cocoa types, the alkaline pretreatment resulted in the lowest yield (p<0.05), with values of (58.24±3.40)% and (37.72±1.57)% for national and CCN-51, respectively. On the other hand, autohydrolysis had the highest yields, with values of (70.69±0.34)% for national cocoa and (50.15±0.24)% for CCN-51. The lower yield levels found on CCN-51 variety may be related to its high lignin content, and this could be explained through the composition of the pretreated biomasses, shown in Figure 3. In the case of national cocoa (Figure 3a), the acid pretreatment presented the highest lignin content (43.14±2.15)%, while the alkaline pretreatment had the lowest lignin percentage, (18.75±3.31)%, and the highest cellulose composition of (57.34±7.29)%. Cellulose content was always higher than in the pretreated biomass, a pattern that was also observed in CCN-51 (Figure 3b). Nonetheless, for the latter, lignin composition, after any pretreatment, was lower than that of the untreated biomass. Once again, the alkaline pretreatment resulted in the highest and lowest lignin and cellulose contents, with values of (24.43±4.01)% and (55.19±1.65)%, respectively. There were no significant differences between the acid treatment and autohydrolysis.
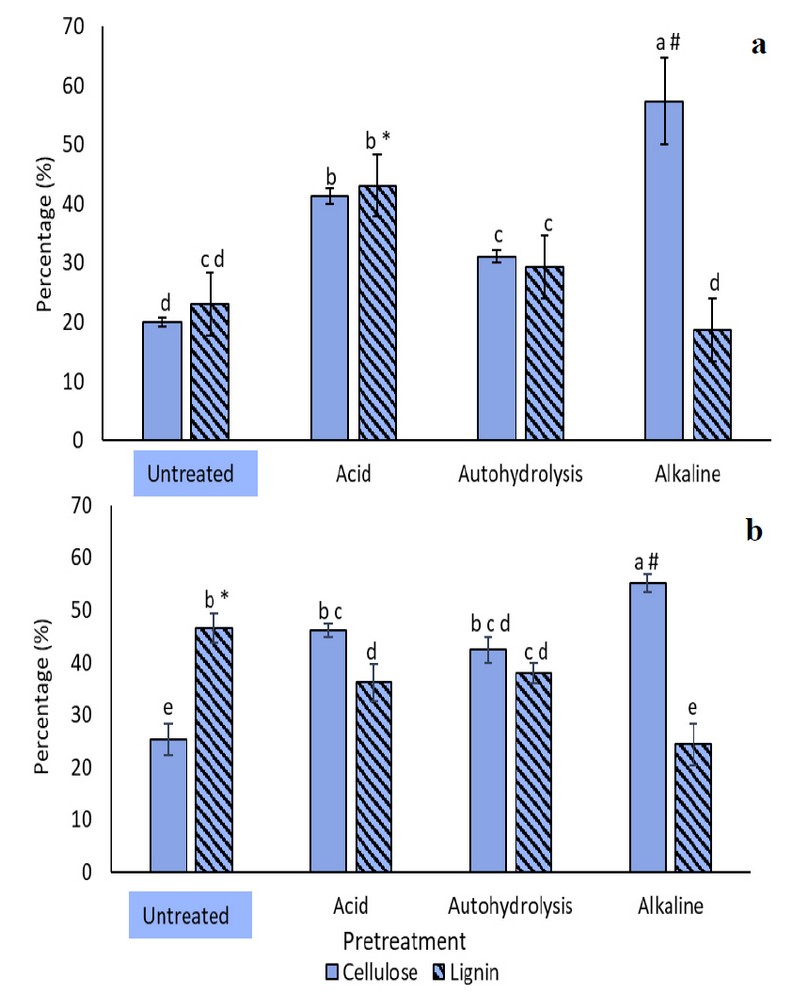
Figure 3. Lignin and cellulose content before and after each pretreatment applied to the husk of a) National cocoa and b) CCN-51 pod. (*) and (#) represent maximum amounts of lignin and cellulose content, respectively (p <0.05). Pretreatments with the same letters do not present statistically significant differences.
As previously mentioned, the pretreatments’ purpose is to depolymerize lignin and, possibly, hemicellulose structures. Furthermore, in this process, part of the cellulose is also hydrolyzed into reducing sugars, useful in subsequent bioprocesses 26. At the same time, the remaining cellulose would be more readily available for enzymatic hydrolysis. The acid hydrolyses hemicellulose and dissolves lignin by breaking glycosidic bonds, which leads to polysaccharides being transformed into oligomeric and monomeric sugars 7. However, the acid concentrations and temperatures used in this work are low compared to others reported in the literature 27,28, and de-lignification is less significant than autohydrolysis, where only water was used.
On the other hand, the alkaline pretreatment breaks the lignin structure by swelling cell walls and reducing its crystallinity, increasing the surface area of cellulose 29. This pretreatment seemed to be more efficient at delignifying both types of biomass, a fact of the high cellulose contents corroborate that. Moreover, the extent of delignification may be responsible for the lower yields observed for the CCN-51 samples. As they have higher lignin contents, and the alkaline pretreatment proved successful at eliminating lignin, the greater mass was lost in the pretreatment of this cocoa type.
Changes in cocoa pod shell morphology after each pretreatment
As biomass composition was altered after pretreatment, it was essential to evaluate morphological changes that could explain, from a physical standpoint, what occurred to the structure 30. Figure 4 shows the micrographs of untreated and treated biomass for both cocoa types.
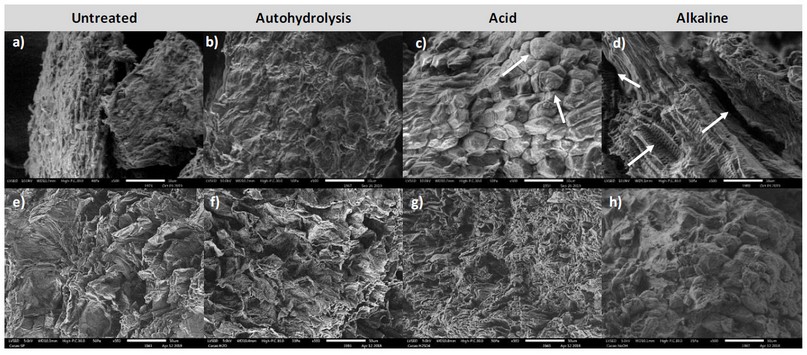
Figure 4. Scanning electron micrographs of pod shell biomass from National Cocoa (top row) and CCN-51 (bottom row) at 500x magnification. Untreated (a,e), autohydrolysis (b,f), acid (c,g) and alkaline (d,h). Arrows indicate cracks and structures resulting from possible biomass degradation. Calibration bar: 50µm.
For national cocoa (Figures 4 a-d), significant changes can be observed after the pretreatments. The untreated biomass had limited porosity and resulted from autohydrolysis (Fig. 4a and b). The acid pretreatment (Fig. 4c) seemed to generate a slight degradation in terms of the biomass structure, as implied by different structures’ appearance (arrows). On the other hand, the biomass after alkaline pretreatment (Fig. 4d) presents a series of pores and cracks (arrows) that could also be a sign of degradation, which may be due to a higher degree of lignin removal, as found in changes in biomass composition (Fig. 3a). It should be taken into account that part of the hemicellulose could also be degraded during the alkaline pretreatment, decreasing the xylan content in the lignocellulosic material, leading to a greater extent of biomass degradation 31.
In CCN-51 (Figures e-f), the changes are less apparent in porosity, but some modifications can be observed on surface roughness. Thus, given the more significant changes in biomass morphology, along with greater de-lignification, and larger yields, National Cocoa was further studied, and thereby, the following results pertain to this type of biomass.
Compositional analyses by FTIR spectra
National cocoa pod shell biomass, both untreated and pretreated, was analyzed by FTIR to corroborate compositional changes, and the resulting spectra are presented in Figure 5, where changes in the presence of peaks and peak intensity took place. In this section, peaks will be mentioned about the untreated biomass, as a shift in the entire spectra was observed for the pretreated samples, a pattern that was reproduced in the laboratory. A small-signal can be found at 1,164 cm-1 due to cellulose C-O-C asymmetric stretching 32; this peak increases in intensity after pretreatments, becoming most visible after the alkaline attack. A similar effect is observed at 830cm-1, which represents cellulose β-glycosidic linkages 33. The band at 1232 cm-1 corresponds to the C-O stretching of hemicellulose and lignin, a signal that significantly decreases in the acid and alkaline samples. Bands associated with skeletal vibrations of aromatic rings within the lignin structure are seen at 1510 and 1680 cm-1 34, with the latter reducing its intensity in the pretreated alkaline sample. The peak at 1732 cm-1 corresponds to ketone and aldehyde vibrations (C=O) from hemicellulose 33, and it can be seen that this signal is maintained after autohydrolysis but significantly reduced by the acid and alkaline treatments.
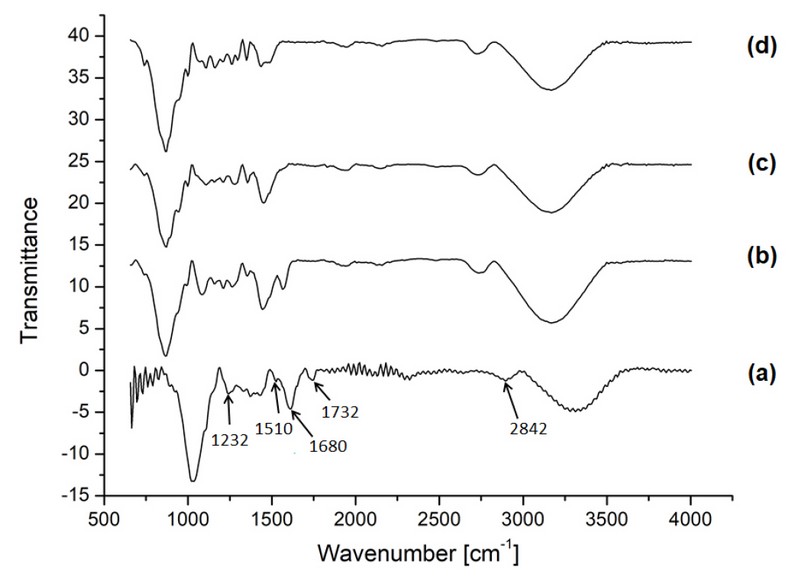
Figure 5. FTIR spectra of the untreated biomass (a) and biomass pretreated under (b) autohydrolysis, (c) acid, and (d) alkaline.
The observations from the spectra corroborate what was found in changes in biomass composition (Figure 3). There is a clear indication of more excellent cellulose content, evidenced by the higher peak intensities after pretreatments, especially in the pretreated alkaline biomass. Similarly, reduction in signals corresponding to the lignin structure is under the de-lignification claimed previously. However, there is new information gathered in Figure 6, as peaks related to hemicellulose also decrease significantly, indicating that all pretreatment conditions produce hemicellulose hydrolysis, as reported by Poletto et al.(2012)35. Pupiales et al. (2020) reported a similar behavior for the alkaline pretreatment of the mesocarp from cocoa pod shell when producing porous sponges for biomedical applications34.
Biomass thermal stability
TGA was performed on untreated and pretreated biomass to observe the changes produced in the biomass’s thermal stability, corroborating changes in its structure and composition.
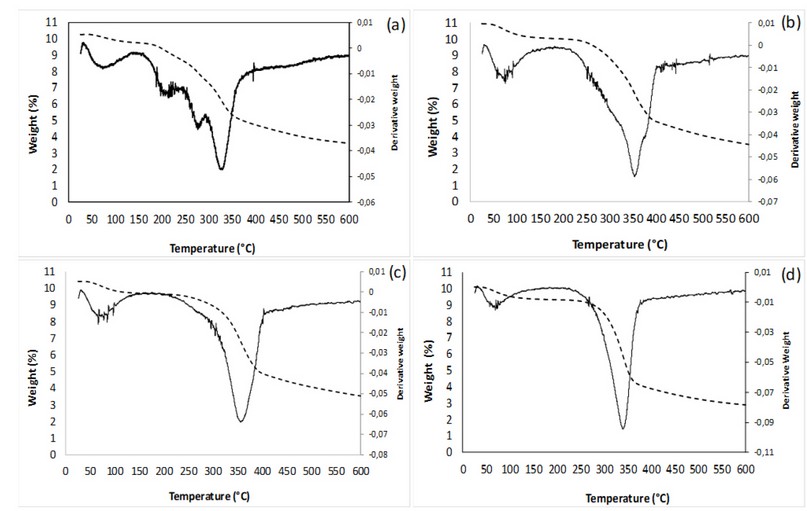
Figure 6. Thermogravimetric analysis for biomass samples a) untreated, b) acid, c) autohydrolysis, and d) alkaline pretreatments. Dotted and solid lines represent weight loss with temperature and its first derivative, respectively.
As seen in Figure 6, the curves of mass loss concerning temperature varied for each sample, with the untreated biomass (Fig. 6a) having a less pronounced slope in the degradation section between 160 and 400ºC. In its first derivative, multiple peaks are seen before reaching the maximum degradation temperature, which may correspond to the sample’s heterogeneity with other components, such as pectin, lipids, polyphenols, and other carbohydrates were not quantified 3. It has been previously reported that the temperature of maximum degradation for pectin is about 250ºC 36. With the treated samples, this effect disappears, and only a peak related to water evaporation remains. Nevertheless, the curves shift, and the initial and maximum degradation temperatures change, as reported in Table 2.
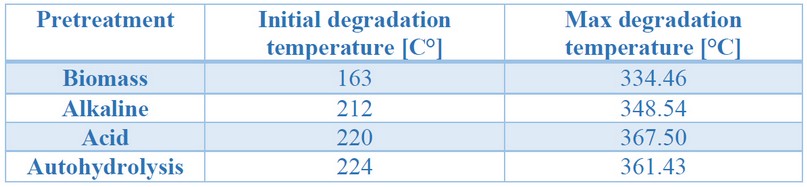
Table 2. Initial and maximum degradation temperatures obtained from the thermogravimetric analysis of untreated and treated biomass
The initial and maximum degradation temperature varied concerning each of the pretreatments; this may be a consequence of the cellulose molecule’s release, a polymer with many strong, crystalline, unbranched glucose units 37. It is observed, in Figure 2, that acidic pretreatments and autohydrolysis have a higher composition of lignin, which is more difficult to degrade, having three stages: the first related to water evaporation, the second, between 200 and 450°C, is due to the evaporation of phenols, and the third, above 400°C, relates to the disintegration of aromatic rings 37. It is within the second stage that the temperature of maximum degradation was observed.
Biomasses resulting from acid and autohydrolysis, which had similar compositions, presented similar values in maximum and initial degradation temperatures. However, those from the alkaline pretreatment, having a lower lignin concentration, and being lignin more difficult to degrade28, presented a lower temperature of maximum degradation and started to degrade earlier. The untreated biomass, on the other hand, presented a different behavior as it is a more heterogeneous matrix, due to the presence of other compounds, previously mentioned, which played a significant role in degradation, which started at the lowest temperature of all the samples, as well as the lowest temperature of maximum degradation. These results follow what was observed in the FTIR analyses, where evidence in lower lignin content for the alkaline treated biomass could result in lower thermal stability. Simultaneously, those from the autohydrolysis and acid treatment were more thermally stable, perhaps due to higher lignin and hemicellulose contents.
Reducing sugars after pretreatments and enzymatic hydrolysis
Once the pretreatment and enzymatic hydrolysis processes had been completed, the concentrations of reducing sugars present in the pretreatment liquors and enzymatic hydrolysates were determined to assess potential uses in bioprocesses. In Figure 7, it can be observed that, after the alkaline pretreatment, the highest sugar concentration of (46,46±1,98) g/L was obtained, while autohydrolysis and the acid pretreatment resulted in similar concentrations between 14 and 15g/L. Except for autohydrolysis, sugar concentration in the hydrolysates was lower than those found in the pretreatment liquors, and those resulting from acid and untreated biomass (not shown) had negligible sugar levels. In this case, the samples from autohydrolysis were hydrolyzed best by the enzyme, with a sugar concentration of (17.21 ± 5.51) g/L.
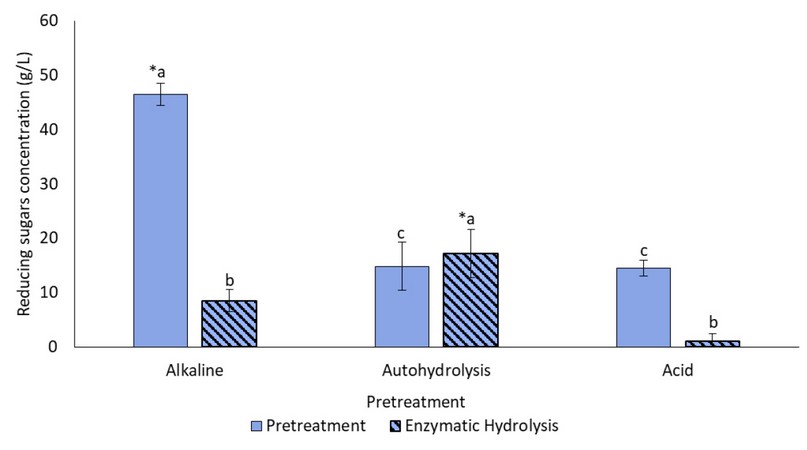
Figure 7. Reducing sugar concentration in each pretreatment liquor and enzymatic hydrolysate. (*) Represents the maximum concentration of the pretreatments (p <0.05). Pretreatments with the same letters do not present statistically significant differences.
Alkali pretreatment is an effective way to remove lignin by causing swelling; it also causes partial hydrolysis of hemicellulose and cellulose 38. This may be the reason for the highest sugar concentration in the alkaline pretreatment liquor. On the other hand, acid pretreatments solubilize lignin and have proven to be efficient at hydrolyzing cellulose and hemicellulose 39. However, most dilute-acid treatments reported in the literature occur at higher temperatures (above 130ºC) than those used in the present study. Thus, the relatively low temperature could have affected this effect, but higher temperatures result in economically unfeasible processes reported by some authors 28. Autohydrolysis had a similar effect to that of the dilute acid, but usually with lower yields40; in this case, given the reduced acid effect due to lower temperatures, it could imply that similar sugar concentrations were obtained after both pretreatments.
But acid treatments pose other vital limitations. During dilute-acid attacks on biomass, important enzyme inhibitors, such as hydroxymethylfurfural (HMF) and the furfural compound, can be generated due to the degradation of pentoses and hexoses 41. This effect is less relevant under alkaline conditions; nonetheless, some authors have reported that, for cellulase to act efficiently on alkaline-pretreated lignocellulose, lignin concentration should be below 18%, lower than the levels achieved for the cocoa pod shell biomass40. Therefore, despite being a suitable method for producing reducing sugars during pretreatment, the alkaline pretreatment herein used is not suitable for subsequent enzymatic hydrolysis. This method should be studied further, modifying processing variables.
Sugar consumption during fermentation of the pretreatment liquors and enzymatic hydrolysates
Despite the important differences observed in reducing sugar concentrations and the potential generation of inhibitory by-products from the pretreatments, it was essential to assess their fermented capacity. Curves of sugar consumption in the fermentation of pretreatment liquors and enzymatic hydrolysates are shown in Figure 8. When fermenting liquors (Fig. 8a), sugars were consumed in a similar trend for all of them, with over 95%.
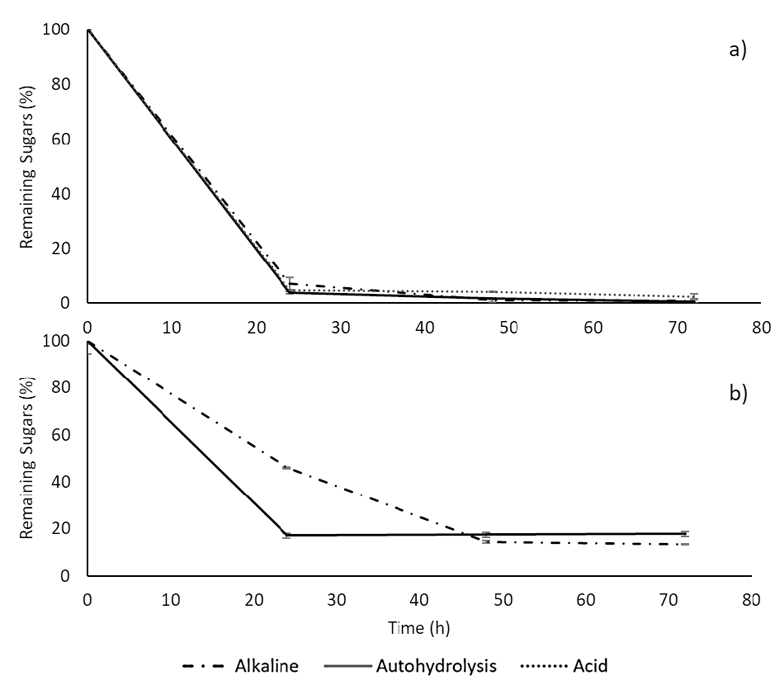
Figure 8. Kinetics of sugar consumption is represented by the percentage of remaining reducing sugars in time, fermentation of a) pretreatment liquors, and b) enzymatic hydrolysates. * Fermentation of the enzymatic hydrolysis was not carried out for the acid pretreatment biomass due to negligible initial sugar concentrations.
During hydrolysate fermentation (Fig. 8b), a different behavior was found. In this case, after 24h, the remaining sugars were (46,10±0,15)% and (17,14±0.30)% for enzymatic hydrolysates of samples from autohydrolysis and alkaline pretreatments, respectively. After 72h, a significant amount of sugars remained (13-17%), implying that yeast growth is slower than in liquor fermentation. The alkaline-pretreated samples could be attributed to the low sugar concentrations after hydrolysis, resulting in insufficient nutrients or the generation of growth inhibitors, such as furan derivatives and polyphenols 42.
Bioethanol production
Bioethanol production was assessed in two different ways, as shown in Figure 9. Productivity (Fig. 9a) would give an insight into the potential large-scale applications of the proposed process since it indicates how much ethanol can be produced from a known amount of biomass, while yield (Fig. 9b) is used as a measure of how efficiently the yeast is metabolizing sugars for ethanol production. There were no statistical differences between productivity for all pretreatment liquors, with values around 28 g of ethanol per kg of untreated biomass, even though initial sugar concentrations were different (Figure 7). After enzymatic hydrolysis, as expected, given the low sugar yields, productivity decreased; in this case, autohydrolysis resulted in the most massive productivity of (20.81±0.14) g EtOH/kg Biomass. Nevertheless, despite having lower productivity, enzymatic hydrolysates displayed higher yield, where, again, the autohydrolysis was the most efficient, as it was also among the pretreatment liquors. For the latter, there were no differences between the liquors resulting from the acid and alkaline pretreatments.
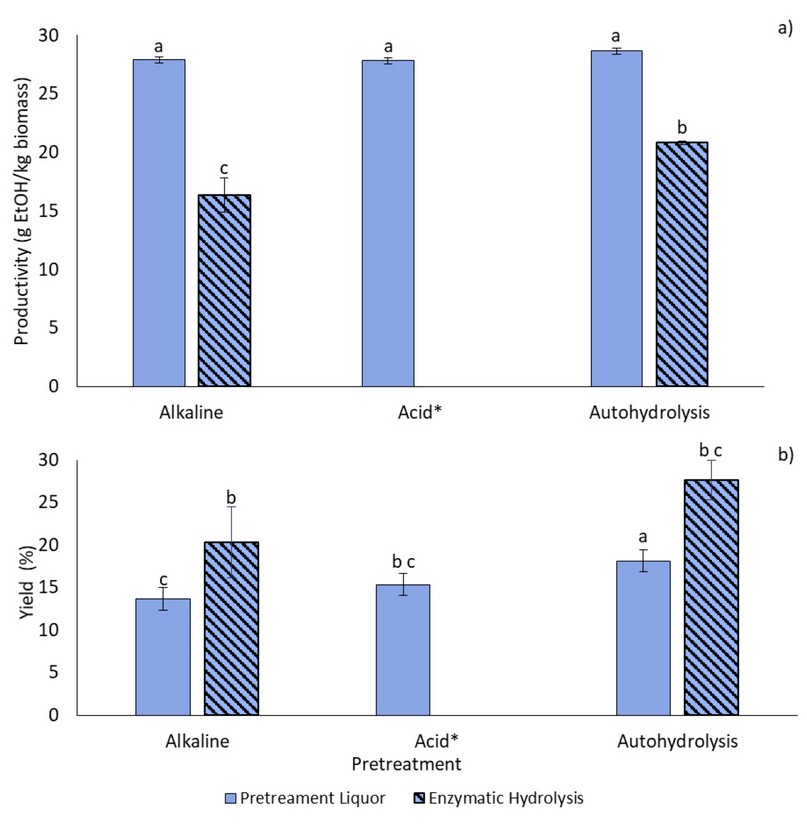
Figure 9. Bioethanol production levels reported as a) Productivity and b) yield of pretreatment liquors fermentation and enzymatic hydrolysates. * Enzymatic hydrolysis fermentation was not carried out for the acid pretreatment due to negligible initial sugar concentrations. Pretreatments with the same letters do not present significant differences.
Productivities and efficiencies obtained in this work were low in comparison to previous studies with other biomasses 4,43. This was expected, particularly for liquors from the acid and autohydrolysis pretreatments, given their low sugar concentrations. However, the fact that alkaline liquors had the same productivity, with sugar concentrations 3-fold from the other pretreatments, may be a sign of inhibition for ethanol production 41. This is corroborated by the yield, which indicates that only up to 18% (autohydrolysis) of the available sugar is converted into ethanol. After enzymatic hydrolysis, this effect is improved, but many sugars are still not converted into ethanol.
Interestingly, these sugars are consumed. It is possible that the yeast strain used in the study was not the most appropriate one. Yeast selection is essential as cells are subjected to different stresses resulting from biomass pre-processing 44. Thus, screening should be conducted to select a yeast with higher short-term productivity.
CONCLUSIONS
The present study was a comprehensive first approximation for valorizing relevant lignocellulosic biomass in Ecuador, cocoa pod shell. Generating reducing sugars from these residues could be useful not only for bioethanol production but for other metabolites of interest, such as organic acids and lipids, among others. To achieve this goal, we have proposed using traditional pretreatment methods to understand this biomass behavior and its potential for further studies. Relatively mild pretreatments, including autohydrolysis and dilute acids and bases, significantly affect biomass composition and properties, particularly reducing lignin content significantly in alkaline conditions. However, this reduction might not be sufficient for cellulase hydrolysis under the studied conditions, and strong indications of enzymatic and bioethanol production inhibition were observed for the alkaline and acid pretreatments. Autohydrolysis appeared to have the lesser inhibition effects, and optimizing these pretreatment conditions could be beneficial for industrial applications as it would have a limited environmental impact. These results are promising for cocoa pod shell valorization, and further investigation is worth exploring
Acknowledgments
The authors are thankful to Sebastian Salazar, from the Department of Chemical Engineering, USFQ, for his help generating a calibration curve for ethanol quantification.
Funding Information
This work has been funded by the Collaboration Grants program at Universidad San Francisco de Quito.
Conflict of interest
The authors declare no conflicts of interest in the present work.
REFERENCES
1. Alcamo J, Moreno B, Nováky M, et al. Europe. Climate Change 2007: Impacts, Adaptation and Vulnerability. Clim Chang 2007 Impacts, Adapt Vulnerability Contrib Work Gr II to Fourth Assess Rep Intergov Panel Clim Chang 2007; 541–580.
2. Carmona-Cabello M, Sáez-Bastante J, Pinzi S, et al. Optimization of solid food waste oil biodiesel by ultrasound-assisted transesterification. Fuel 2019; 255: 115817.
3. Althuri A, Gujjala LKS, Banerjee R. Partially consolidated bioprocessing of mixed lignocellulosic feedstocks for ethanol production. Bioresour Technol 2017; 245: 530–539.
4. Wei H, Yingting Y, Jingjing G, et al. Lignocellulosic Biomass Valorization: Production of Ethanol. Elsevier. Epub ahead of print 2017. DOI: 10.1016/B978-0-12-409548-9.10239-8.
5. de Barros R da RO, Becarelli P, de Oliveira RA, et al. Triticum spelta straw hydrothermal pretreatment for the production of glucose syrups via enzymatic hydrolysis. Biochem Eng J 2019; 151: 107340.
6. Mosier N, Wyman C, Dale B, et al. Features of promising technologies for pretreatment of lignocellulosic biomass. Bioresour Technol 2005; 96: 673–686.
7. Solarte-Toro JC, Romero-García JM, Martínez-Patiño JC. Acid pretreatment of lignocellulosic biomass for energy vectors production : A review focused on operational conditions and techno-economic assessment for bioethanol production. Renew Sustain Energy Rev 2019; 0–1.
8. Lee S-M, Kim KH, Ko JK, et al. Ethanol production from lignocellulosic hydrolysates using engineered Saccharomyces cerevisiae harboring xylose isomerase-based pathway. Bioresour Technol 2016; 209: 290–296.
9. Hama S, Kihara M, Noda H, et al. Development of cell recycle technology incorporating nutrient supplementation for lignocellulosic ethanol fermentation using industrial yeast Saccharomyces cerevisiae. Biochem Eng J 2018; 137: 23–29.
10. Jiang D, Hao M, Fu J, et al. Potential bioethanol production from sweet sorghum on marginal land in China. J Clean Prod 2019; 220: 225–234.
11. ANECOCOA. Exportación Ecuatoriana De Cocoa – 2015 Exportación Ecuatoriana De Cocoa – 2015. Asoc Nac Export Cocoa 2015; 6p.
12. Espinoza Guzman R, Albornoz Vintimilla E, Medina Palacios S. Atlas Bioenergético del Ecuador. Quito, 2014.
13. Ardila C, Carreño S. Aprovechamiento de la cáscara de la mazorca de cocoa como adsorbente. Univ Ind Santander 2011; 13: 234.
14. Mateus, Lady, Hernandez O, Velasquez M, et al. Evaluación del pretratamiento con ácido sulfúrico diluido del pasto maralfalfa (Pennisetum glaucum x Pennisetum purpureum) para la producción de etanol. Rev Colomb Biotecnol 2012; 14: 146–156.
15. Karapatsia A, Pappas I, Penloglou G, et al. Optimization of Dilute Acid Pretreatment and Enzymatic Hydrolysis of Phalaris aquatica L. Lignocellulosic Biomass in Batch and Fed-Batch Processes. Bioenergy Res 2017; 10: 225–236.
16. Larrea FA, Salazar S, Andino C, et al. comparison of bioethanol production of starches from different andean tubers. Chem Eng Trans 2020; 80: 259–264.
17. First Action 1934 Final Action. Comp A J Comp Educ 1934; 577: 6155–6155.
18. AOAC. AOAC Official Method 920.39. AOAC Off Methods Anal 2002; 888–898.
19. Thiex N. Evaluation of analytical methods for the determination of moisture, crude protein, crude fat, and crude fiber in distillers dried grains with solubles. J AOAC Int 2009; 92: 61–73.
20. Lynch JM, Barbano DM. Kjeldahl nitrogen analysis as a reference method for protein determination in dairy products. J AOAC Int 1999; 82: 1389–1392.
21. Domínguez-Domínguez MM, Álvarez-Castillo A, Granados-Baeza M, et al. Estudio de la cinética del pretatramiento e hidrólisis ácida del bagazo de caña de azúcar. Iberoam Polim 2012; 13: 200–211.
22. Gusakov A V., Kondratyeva EG, Sinitsyn AP. Comparison of Two Methods for Assaying Reducing Sugars in the Determination of Carbohydrase Activities. Int J Anal Chem 2011; 2011: 1–4.
23. Campos-Vega R, Nieto-Figueroa KH, Oomah BD. Cocoa (Theobroma cocoa L.) pod husk: Renewable source of bioactive compounds. Trends Food Sci Technol 2018; 81: 172–184.
24. Han X, Bao J. General Method to Correct the Fluctuation of Acid Based Pretreatment Efficiency of Lignocellulose for Highly Efficient Bioconversion. ACS Sustain Chem Eng 2018; 6: 4212–4219.
25. Alvarez-Barreto JF, Quintero D, Rodríguez M, et al. Evaluation of phenolic compounds, lignin, amino acids and carbohydrates in theobroma cocoa L. From three different climate regions in venezuela. Emirates J Food Agric 2018; 30: 522–530.
26. Mahmood H, Moniruzzaman M, Iqbal T, et al. Recent advances in the pretreatment of lignocellulosic biomass for biofuels and value-added products. Curr Opin Green Sustain Chem. Epub ahead of print 2019. DOI: 10.1016/j.cogsc.2019.08.001.
27. Zheng Y, Zhao J, Xu F, et al. Pre-treatment of lignocellulosic biomass for enhanced biogas production. Progress in Energy and Combustion Science 2014; 42: 35–53.
28. Abraham A, Mathew AK, Park H, et al. Pretreatment strategies for enhanced biogas production from lignocellulosic biomass. Bioresour Technol 2020; 301: 122725.
29. Sun S, Cao X, Sun S, et al. Improving the enzymatic hydrolysis of thermo-mechanical fiber from Eucalyptus urophylla by a combination of hydrothermal pretreatment and alkali fractionation. Biotechnol Biofuels 2014; 7: 1–12.
30. Zeng M, Ximenes E, Ladisch MR, et al. Tissue-specific biomass recalcitrance in corn stover pretreated with liquid hot-water: SEM imaging (part 2). Biotechnol Bioeng 2012; 109: 398–404.
31. Lin YS, Lee WC, Duan KJ, et al. Ethanol production by simultaneous saccharification and fermentation in rotary drum reactor using thermotolerant Kluveromyces marxianus. Appl Energy 2013; 105: 389–394.
32. Dávila I, Gullón P, Labidi J, et al. Assessment of the influence of the temperature in the microwave-assisted alkaline delignification of vine shoots. Chem Eng Trans 2018; 70: 1687–1692.
33. Sangian HF, Pangau JR, Tamuntuan GH, et al. The structural analysis of the lignocellulose, champaca timber (Elmerrilliaovalis) modified by the microwave. Chem Eng Trans 2018; 65: 229–234.
34. Pupiales B, Galeas S, Guerrero V, et al. generation of porous scaffolds from cocoa mesocarp for biomedical applications using surface response methodology. Chem Eng Trans 2020; 79: 151–156.
35. Poletto M, Pistor V, Santana RMC, et al. Materials produced from plant biomass. part II: Evaluation of crystallinity and degradation kinetics of cellulose. Mater Res 2012; 15: 421–427.
36. Almeida EAMS, Facchi SP, Martins AF, et al. Synthesis and characterization of pectin derivative with antitumor property against Caco-2 colon cancer cells. Carbohydr Polym 2015; 115: 139–145.
37. Diaz AB, Moretti MM de S, Bezerra-Bussoli C, et al. Evaluation of microwave-assisted pretreatment of lignocellulosic biomass immersed in alkaline glycerol for fermentable sugars production. Bioresour Technol 2015; 185: 316–323.
38. Kim JS, Lee YY, Kim TH. A review on alkaline pretreatment technology for bioconversion of lignocellulosic biomass. Bioresour Technol 2016; 199: 42–48.
39. Lorenci Woiciechowski A, Dalmas Neto CJ, Porto de Souza Vandenberghe L, et al. Lignocellulosic biomass: Acid and alkaline pretreatments and their effects on biomass recalcitrance – Conventional processing and recent advances. Bioresource Technology 2020; 304: 122848.
40. Kumar P, Barrett DM, Delwiche MJ, et al. Methods for pretreatment of lignocellulosic biomass for efficient hydrolysis and biofuel production. Industrial and Engineering Chemistry Research 2009; 48: 3713–3729.
41. Singh B, Kumar P, Yadav A, et al. degradation of fermentation inhibitors from lignocellulosic hydrolysate liquor using immobilized bacterium, Bordetella sp. BTIITR. Chem Eng J 2019; 361: 1152–1160.
42. Kim Y, Ximenes E, Mosier NS, et al. Soluble inhibitors/deactivators of cellulase enzymes from lignocellulosic biomass. Enzyme Microb Technol 2011; 48: 408–415.
43. Guigou M, Cabrera MN, Vique M, et al. Combined pretreatments of eucalyptus sawdust for ethanol production within a biorefinery approach. Biomass Convers Biorefinery 2019; 9: 293–304.
44. Kumari R, Pramanik K. Improvement of multiple stress tolerance in yeast strain by sequential mutagenesis for enhanced bioethanol production. J Biosci Bioeng 2012; 114: 622–629.
Received: 25 October 2020
Accepted: 20 November 2020
1Jose F. Alvarez-Barreto*, 1 Fernando Larrea, 1 Maria C. Pinos C, 1 Jose Benalcázar, 1 Daniela Oña, 1Daniela A. Viteri, 2Marco Leon, 1Daniela Almeida-Streitwieser D.
1 Institute for the Development of Alternative Energies and Materials, IDEMA. Department of Chemical Engineering, Universidad San Francisco de Quito, Ecuador.
2 Institute for Materials Research and Applications, IIMA. Department of Mechanical Engineering. Universidad San Francisco de Quito, Ecuador.
* Corresponding autor:
Valle de Cumbayá, Diego de Robles S/N y vía Interoceánica. Campus Cumbayá
Edificio Hayek, Ofic. H320. Quito, Ecuador
Email: jalvarezb@usfq.edu.ec
Tel: +593-2971700 ext. 1328